QUESTIONS FOR ENERGY DEMOCRACY:
What are the physical realities that any energy system needs to deal with, regardless of how we organise its rules, ownership, and goals?
This guide aims to help us to re-imagine how our energy systems could be organised. To do that, we need to understand what is and isn’t changeable, starting with some fundamental physical realities.
Electricity is a particularly important form of energy for a sustainable energy system – sometimes so much so that when people say ‘energy’ they are actually talking only about electricity. Because of this, this guide includes a separate section on the Physics of Electricity that goes into more detail.
What is energy?
The understanding of ‘energy’ as a single concept was developed during the industrial revolution, starting from about 1680. It covers different physical phenomena including heat, motion, electricity and light. We get this energy from coal, wood or dung, water stored in a reservoir or flowing in a river, the wind, the sun.
Using the concept of ‘energy‘ to include all of these phenomena is central to industrial societies. It allows us to apply the same scientific units and equations to all forms of energy, and to understand the conversion between different forms in terms of the first and second laws of thermodynamics. This is particularly important for designing and running thermal power stations and networked energy infrastructure.
For a critical discussion of the invention of ‘energy’ as a unified concept, see this essay by teacher Robert Lehrman, or this report by the cornerhouse which puts the history of ‘energy’ in a social and political context.
The interchangeable concept of energy is illustrated by definitions of energy such as ‘the ability to heat water’ or ‘the ability to do work’, both important functions in the context of the industrial revolution.
During the industrial revolution, chemists and physicists were studying the combustion of fuels including coal, wood and gas. They wanted to compare the amount of coal burned to heat a certain amount of water from 15 degrees to 100 degrees is with the amount of wood or gas needed to heat the same volume of water by the same temperature difference. They developed a unit to define this ‘ability to heat water’, the calorie, often abbreviated to cal.
At the same time, physicists and engineers were studying moving objects, such as trains, pistons and levers. To compare different ways of moving objects, they created the concept of ‘the ability to do work’. This is a particular definition of ‘work’ used in physics, meaning a force causing movement of an object. The quantity of work is simply the force times the distance travelled. This is measured in Joules.
Steam engines turn combustion of fuel into movement of objects, and so the two systems of understanding were brought together, and understood to be the same physical quantity: energy.
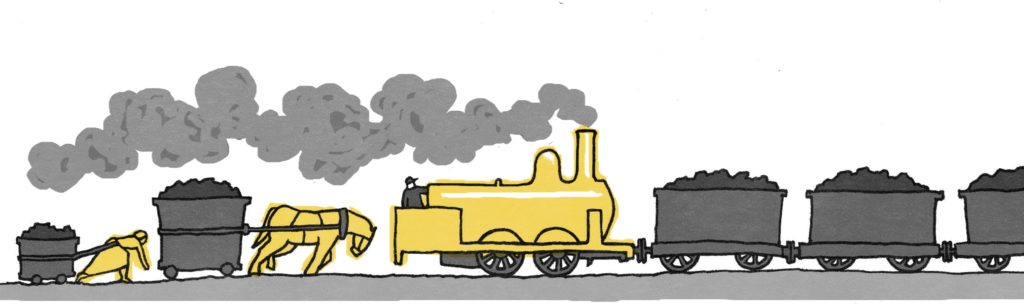
This connection of heat and movement, through the concept of energy, is called thermodynamics (meaning the study of heat – ‘thermo’ – and movement – ‘dynamics’) .
The single concept of ‘energy’ is used to include:
Electricity
The movement of electrons in a conducting material
Kinetic energy
Energy in movement, (e.g. the turning of a wind turbine)
Nuclear energy
The energy released when an atomic nucleus is split, with a rearrangement of subatomic particles
Thermal energy or heat
The vibration or movement of atoms and molecules (kinetic energy at a micro scale!)
Gravitational potential energy
The energy released if a mass that is high up is dropped (e.g. hydroelectric power from water falling from high to low)
Electromagnetic radiation
Radio waves, microwaves, infrared, visible light, ultraviolet, x-rays, and gamma radiation
Chemical energy
The energy that is released when chemical bonds between atoms break or form (e.g. burning a fuel, storing energy in a battery, respiration in plants and animals)
You can’t get anything for nothing: conservation of energy and the first law of thermodynamics
Energy can be transformed or converted from one form to another, but it can never be created or destroyed. Just as a rock can be smashed into sand, then compressed into sandstone, and the amount of matter remains the same at each stage (if it’s a closed system, you can’t lose any sand), energy can be converted from chemical energy in a lump of coal, to heat, to movement, and at each stage the total quantity of energy remains the same.
This is also known as the first law of thermodynamics, or the law of conservation of energy.
This rule also applies when we are converting energy in a power station. Sometimes it can seem like we ‘lose’ energy, but this is usually because some energy is converted to heat, which dissipates into the surroundings. Just as any sand that gets scattered before becoming a rock still exists somewhere in some form. We usually say that this energy is ‘lost’, because we can no longer use it, but that doesn’t mean it has completely disappeared!
You can’t even break even: the second law of thermodynamics or the law of ever-growing mess
It takes much less effort to make a mess than to tidy it – that is why they say “a woman’s work (of cleaning) is never done”. It is easier to produce disorder, or disorganisation, than it is to produce order, or organisation. This is quantified in chemistry and physics as ‘entropy‘ – the more mess, the higher the level of entropy.
Although the total quantity of energy in the world is always conserved, the quality, or level of organisation of the energy is always reduced when it is transformed. This means that the ‘ability to do work’ is reduced after every transformation – some of the ‘useful energy’ is lost as heat.
This is the second law of thermodynamics.
Gravitational potential energy is a highly ordered form of energy – a mass, held at a height, waiting to fall down the hill. Heat, on the other hand is very disordered – many molecules are all travelling in different directions. You can convert all oft he gravitational potential energy to heat, but you can’t convert all the heat to gravidational potential energy – it’s a one way street.
It is not possible to get useful work out of all of the primary energy that we consume. Even when what we want is heat, we want that heat to be in a particular place (e.g. in a house), and some of it will always escape. Technology and innovation can reduce the amount of loss, or waste, but can never completely eliminate it.
Any energy conversion technology with no losses would be a perpetual motion machine – a physical impossibility.
More on the 2nd law of thermodynamics – deep dive
Primary energy, energy converters, energy carriers (or vectors) and energy storage
It is helpful to distinguish between primary sources of energy (e.g. fossil fuels, solar energy, wind); energy converters (e.g. wind turbine, coal power station), energy carriers, also known as energy vectors, such as electricity, heat or fuel; and energy storage.
Fuels (e.g. coal, gas, wood, oil) are particularly useful as they can act in three different roles: primary energy, vectors and storage – for example natural gas is a form of primary energy, but it can be stored as gas in a tank (storage), and can be piped around the country (vector/carrier). This means that fewer conversions are needed – and remember every conversion comes with a loss (2nd law of thermodynamics). However, the losses from converting a fuel to electricity can be very high.
[new illustration here]
All usable energy sources come from a limited number of primary sources: the sun’s radiation, the gravitational interaction of the sun and moon with the Earth, geothermal heating, and nuclear processes on Earth. Our main source of energy has been fossil fuels, but ultimately these came from the deposits of organic matter produced by prehistoric plants and animals, which used photosynthesis, and ultimately this is energy from the sun. So when we burn fossil fuels, we are burning many years’ worth of buried sunshine. The fossil fuels burned in 1997 would have needed 400 times all of the biological matter produced each year now to be produced. Wind power comes from air movement caused by the sun heating the atmosphere, and waves are caused by wind over the oceans, so when we use wind, wave or solar power we are using current sunshine – at a sustainable rate rather than tapping into many years worth of saved energy.
Primary energy is converted by energy converters such as wind turbines, solar PV panels, or power stations into directly useful forms or transportable forms, including electricity, heat or hydrogen.
Energy carriers or vectors enable energy to be transported from one place to another. Electricity and hydrogen are energy vectors rather than primary sources of energy. Some primary sources of energy are also energy vectors as they can be directly transported (e.g. shipping coal or pumping oil or gas through pipelines).
We need more energy at certain times than others, and some forms of primary energy are only available at certain times. This means that energy needs to be stored. Electricity can only be stored as electricity on a small scale, in a device known as a capacitor. Use of batteries for storing electricity actually means converting electricity to chemical energy and back again (see physics of electricity page). Fuels such as wood, coal or oil can readily be stored. Heat can be stored to some extent e.g. in insulated tanks of hot water, but some is lost (dispersed to the environment) over time.
For more examples of primary energy, storage, conversion and vectors, go to deep dive
Efficiency
Efficiency is a ratio. That means it is a comparison of how much you get of something desirable compared to the cost. When it comes to energy efficiency, this is fairly clear. Economic efficiency, on the other hand, has a lot of hidden value judgments wrapped up in it.
Given that there is always a loss in useful energy of any conversion process, it is useful to be able to quantify how good a process is at converting energy from the form that we have, to a form that we want. Efficiency is the measure of how much we get of what we wanted, relative to what we put in. In a 100% efficient process, we would get out the same effort as we put in, but we’ve already seen this is physically impossible.
For some energy efficiency equations, go to the deep dive
Economists like to use the term ‘efficiency’ as though this was an absolute thing with no ambiguity. However, the term efficiency is meaningless if we do not define what the ‘useful’ outcomes are, and what the ‘total inputs’ are. The useful outputs measured by one person might be different to those measured by another. Similarly, the inputs measured by one person might be different to those measured by another (is unpaid care work including cooking and cleaning included in the ‘cost of labour’?).
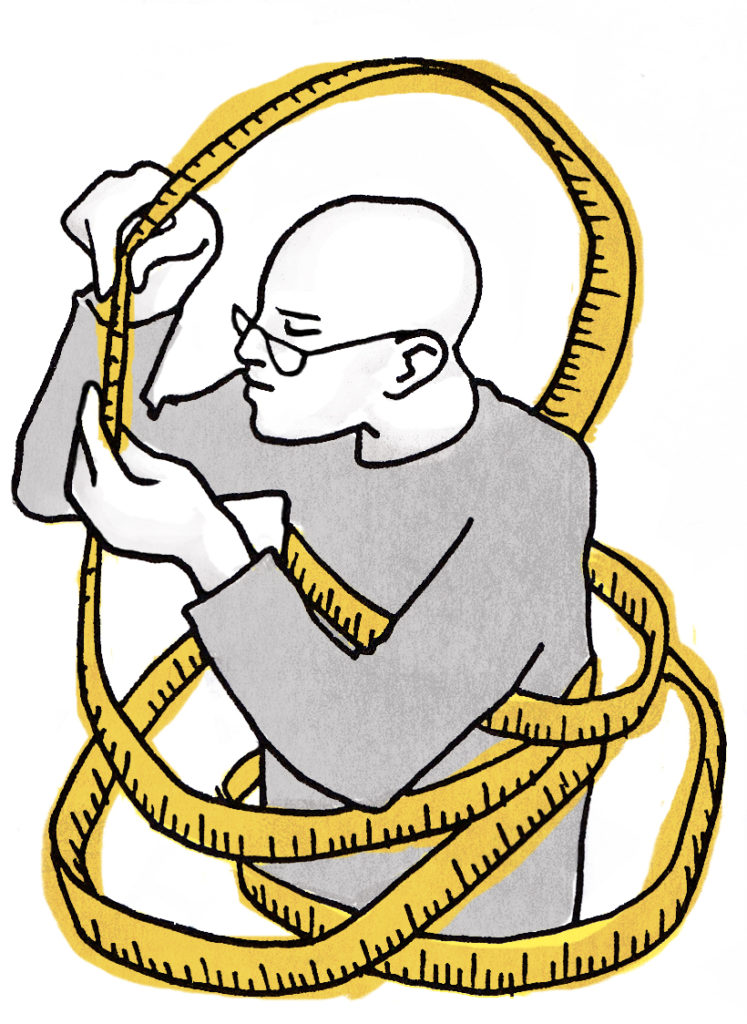
Increasing energy efficiency is sometimes assumed to automatically lead to a reduction in demand. However, it can lead to using more energy in total. It is worth thinking about a bit more carefully, as this is not always the panacea it seems to be.
Jevons Paradox
When we make an efficiency gain, the benefit of that gain does not necessarily go to reduced overall consumption. For example, if my heating system becomes more energy efficient, I may make my house warmer or keep the heating on more of the time. Alternatively I may use the money I saved on heating to drive across the country for the weekend. This is called a ‘rebound effect’, or ‘Jevons paradox’, where an efficiency gain leads to an increase in consumption.
Jevons first talked about this idea when he realised that making steam engines more efficient would increase the use of coal rather than decrease it – which is what happened. Focusing on ‘energy efficiency’ with the assumption that this will lead to a reduction in total energy demand is therefore naive – but is the main approach taken by a lot of government policy on the ‘demand side’ because our lifestyles are seen as politically ‘non-negotiable’, as was discussed in the social value of energy chapter.
Another way of looking at efficiency gains is a concept that Bruce Lankford calls the ‘paracommons‘. He maps out potential destinations for efficiency gains: the original proprietor; neighbours; nature; the wider economy. What would we need to put in place to ensure that energy efficiency gains actually go to the climate rather than to increased consumption somewhere else?
Primary energy sources and Energy Return on Energy Invested
UK energy statistics are often presented in terms of primary energy and final energy, where primary energy is the raw fuel arriving into the system, and final energy is the useful energy used by households and industry. The difference is the energy ‘lost’ in the energy industry itself, e.g. heat rejected in cooling towers in thermal power stations or heat lost in electricity transmission cables.
A good way of depicting the losses in the energy system, from ‘primary’ energy sources to the useful energy provided to consumers, is a sankey diagram, or flow diagram. The sankey diagram for the UK energy system in 2017 is shown below:
On the left, primary energy inputs enter the energy system. On the right, final energy uses are shown. Energy lost is shown exiting the UK system at the bottom of the diagram. The thickness of each line of flow represents the amount of energy. According to the first law of thermodynamics, total energy should be conserved – i.e. the sum of the final energy on the right, and the lost energy at the bottom, should equal the incoming energy on the left.
There are also energy losses that take place before the energy enters the sankey diagram – the fuel burned in ships and mining machinery, cooling and compression of natural gas for transport as liquefied natural gas or LNG, exploration, drilling and pumping of oil, manufacture of solar panels and wind turbines. There are also losses after the ‘useful’ energy exits the diagram – e.g. ‘waste heat’ from a phone charger etc.
We can calculate a ratio of the final energy available to the energy used to make it available – this is called the ‘energy return on energy invested’, or EROI. If a form of energy has an EROI of 1, that would mean that all of the energy produced is used in production – i.e. it is completely useless. If it has an EROI of 30, that means that 30 times the energy is produced as is used in production, so it’s providing a lot of energy value to society at low energy cost. Fossil fuels have a very high EROI, of the order of 30:1, compared to biomass and wood of the order of 4:1. Wind power has an EROI of about 18:1.
All economic processes use energy, so the availability of abundant energy at low energy cost supports high levels of economic growth. In addition to measuring the EROI of particular energy sources, one can measure the ‘societal EROI’ – the average EROI of a society. Studies show strong correlation between EROI and economic growth. The flows of energy in society are so important that they are taken as an underlying basis for some important heterodox (non-mainstream) schools of economic thought, as are land and labour.
The use of fossil fuels during the 19th and 20th centuries enabled high levels of economic growth. The EROI of unconventional fossil fuels, e.g. shale oil or shale gas, is much lower than conventional fossil fuels (around 4:1 for Canadian tar sands), and even the EROI of conventional fossil fuels has reduced dramatically over time (global oil and gas in 1999 EROI 35, in 2006 was 18), as the best resources were used first.
Additionally, renewable energy sources from wind and sun are reliant on energy being stored. If the energy needed to create the storage is also included, this reduces the EROI of those forms of energy. The EROI of various fuels and renewable energy sources, with and without storage is shown in the image below.
The exact methodology used for measuring EROI is important, particularly as the boundary of what is included can be set at different points (e.g. do you count the energy used to build the roads that the oil tankers drive on). Additionally, some EROI calculations take into account the quality of the final energy. If we measure the energy needed to produce electricity from different primary energy sources, we get a very different picture.
The image below shows the EROI for the thermal energy available from different fuels
The following image shows the EROI for electric energy available. The high losses in converting coal to electricity means that coal and gas have a lower EROI for electricity than wind and solar PV. This does not take into account the energy used for storage.
Things to note about EROI
- If future societal EROI is going to be lower than EROI in the last 2 centuries, this has potentially serious implications for economic growth. This is being explored by the Transition Towns movement, the degrowth movement, and studies of prosperity without growth.
- It is good to think about how much energy is needed to produce energy, rather than just how much it costs financially (although these are often correlated)
- EROI is just one measure – the amount of land used to produce energy is also extremely important, as are other issues (including climate change, biodiversity, social impact etc). Some of these may be correlated with EROI.
- The assumptions and boundaries of what is included make a big difference to the outcomes
Power – energy used over time
Often, it is useful to know the rate at which energy is used or transferred – how much electricity can a power station produce per second? How quickly can a car engine transfer enough energy from petrol to accelerate the car from zero to 60? How fast does the heat leave a warm house on a cold day? How bright is a light bulb? This is called power, a measure of the rate of transfer of energy. It is measured in a unit of Watts (W), which the number of joules transferred per second.
Another way of thinking about the difference between energy and power, is by analogy with distance and speed. Achieving a task might require a certain amount of energy, and the time taken to complete the task depends on how fast that energy is deployed, or the power. Similarly, to get from one place to another means travelling a certain distance, but the time taken to get to the destination depends on the speed you travel at.
More on power – deep dive
The kWh – our main unit of energy
So far, we have talked about the basic unit of energy, the Joule, and the basic unit of power, the Watt, which is equal to one Joule per second. However, if we measured our electrical energy in Joules all the time we would have to use very large numbers! When measuring amounts of energy consumed in households, it is commonplace to use a unit of energy derived from units of power: the kWh. kWh stands for kilowatt hour, or kW x hours. If we ran our 2kW kettle continuously for 1 hour it would then consume 2kWh of electricity. Although we wouldn’t run a kettle for an hour, the kWh is a convenient measure of the energy consumed by everyday electrical and heating appliances.
This guide uses the kWh as the main unit of energy.
There are many different units of energy, just as there are metric and imperial systems of measuring mass and distance (kilogram vs pound, meter vs yard…). Other units of energy that are commonly used in the context of energy systems include:
barrel of oil equivalent (boe) = 1628.2 kWh (not surprisingly, this comes from the amount of energy contained in a barrel of oil)
British Thermal Unit (btu) = 0.000293 kWh (1000btu = 0.293kWh)
kilotonnes of oil equivalent (ktoe) = 11,630,000 kWh [11.63 GWh]
You might find these units in government energy statistics. All of these different units make comparison tricky, which can be annoying. It is easy to convert between different units with a calculator or using an online unit conversion website such as unitjuggler.
More on units and energy used by household appliances – deep dive
LEARNING POINTS FOR ENERGY DEMOCRACY:
There are many forms of energy, including heat, motion, electricity and stored energy in fuels, and energy can be converted from one form to another. Every time energy is converted from one form to another, transported, or stored, some of it is ‘lost’ to waste heat. This means that however efficient we get, there will be limits to how much useful energy we can get per unit of primary energy. Efficiency is the amount of useful energy you get out divided by the total amount of energy that you put in. Increases in efficiency don’t necessarily lead to reductions in consumption – the Jevons paradox shows that ‘rebound’ can happen and consumption can go up. All energy available originates from the sun, from gravity, or from nuclear reactions, and fossil fuels use many millions of years’ worth of buried sunshine. Energy and power are different – by analogy with water flowing into a bucket, energy is like the amount of water in the bucket, and power is the rate at which the water flows into the bucket. Or energy is like a distance travelled, and power is like the speed at which you travel.
Next: phycics of electricity OR skip to physical infrastructure